An integrated approach identifies the molecular underpinnings of murine anterior visceral endoderm migration
- PMID: 38843837
- PMCID: PMC11511681
- DOI: 10.1016/j.devcel.2024.05.014
An integrated approach identifies the molecular underpinnings of murine anterior visceral endoderm migration
Abstract
The anterior visceral endoderm (AVE) differs from the surrounding visceral endoderm (VE) in its migratory behavior and ability to restrict primitive streak formation to the opposite side of the mouse embryo. To characterize the molecular bases for the unique properties of the AVE, we combined single-cell RNA sequencing of the VE prior to and during AVE migration with phosphoproteomics, high-resolution live-imaging, and short-term lineage labeling and intervention. This identified the transient nature of the AVE with attenuation of "anteriorizing" gene expression as cells migrate and the emergence of heterogeneities in transcriptional states relative to the AVE's position. Using cell communication analysis, we identified the requirement of semaphorin signaling for normal AVE migration. Lattice light-sheet microscopy showed that Sema6D mutants have abnormalities in basal projections and migration speed. These findings point to a tight coupling between transcriptional state and position of the AVE and identify molecular controllers of AVE migration.
Keywords: anterior visceral endoderm; cell migration; embryonic patterning; mouse embryogenesis; phosphoproteomics; semaphorin signaling; single-cell transcriptomics.
Copyright © 2024 The Authors. Published by Elsevier Inc. All rights reserved.
Conflict of interest statement
Declaration of interests The authors declare no competing interests.
Figures
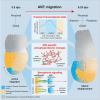
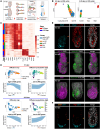
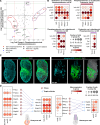
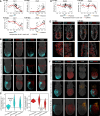
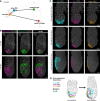
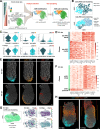
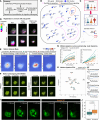
Similar articles
-
Rac1-dependent collective cell migration is required for specification of the anterior-posterior body axis of the mouse.PLoS Biol. 2010 Aug 3;8(8):e1000442. doi: 10.1371/journal.pbio.1000442. PLoS Biol. 2010. PMID: 20689803 Free PMC article.
-
Nodal dependent differential localisation of dishevelled-2 demarcates regions of differing cell behaviour in the visceral endoderm.PLoS Biol. 2011 Feb;9(2):e1001019. doi: 10.1371/journal.pbio.1001019. Epub 2011 Feb 22. PLoS Biol. 2011. PMID: 21364967 Free PMC article.
-
Multi-cellular rosettes in the mouse visceral endoderm facilitate the ordered migration of anterior visceral endoderm cells.PLoS Biol. 2012 Feb;10(2):e1001256. doi: 10.1371/journal.pbio.1001256. Epub 2012 Feb 7. PLoS Biol. 2012. PMID: 22346733 Free PMC article.
-
Heading forwards: anterior visceral endoderm migration in patterning the mouse embryo.Philos Trans R Soc Lond B Biol Sci. 2014 Dec 5;369(1657):20130546. doi: 10.1098/rstb.2013.0546. Philos Trans R Soc Lond B Biol Sci. 2014. PMID: 25349454 Free PMC article. Review.
-
The Head's Tale: Anterior-Posterior Axis Formation in the Mouse Embryo.Curr Top Dev Biol. 2018;128:365-390. doi: 10.1016/bs.ctdb.2017.11.003. Epub 2017 Dec 8. Curr Top Dev Biol. 2018. PMID: 29477169 Review.
Cited by
-
Tissue-intrinsic beta-catenin signals antagonize Nodal-driven anterior visceral endoderm differentiation.Nat Commun. 2024 Jun 13;15(1):5055. doi: 10.1038/s41467-024-49380-0. Nat Commun. 2024. PMID: 38871742 Free PMC article.
References
-
- Gardner R.L., Rossant J. Investigation of the fate of 4–5 day post-coitum mouse inner cell mass cells by blastocyst injection. J. Embryol. Exp. Morphol. 1979;52:141–152. - PubMed
-
- Lawson K.A., Wilson V. In: Kaufman's Atlas of Mouse Development Supplement. Baldock R., Bard J., Davidson D.R., Morriss-Kay G., editors. Vol. 3. Academic Press; 2016. A revised staging of mouse development before organogenesis; pp. 51–64. - DOI
MeSH terms
Substances
LinkOut - more resources
Full Text Sources