Towards engineering carboxysomes into C3 plants
- PMID: 26867858
- PMCID: PMC4970904
- DOI: 10.1111/tpj.13139
Towards engineering carboxysomes into C3 plants
Abstract
Photosynthesis in C3 plants is limited by features of the carbon-fixing enzyme Rubisco, which exhibits a low turnover rate and can react with O2 instead of CO2 , leading to photorespiration. In cyanobacteria, bacterial microcompartments, known as carboxysomes, improve the efficiency of photosynthesis by concentrating CO2 near the enzyme Rubisco. Cyanobacterial Rubisco enzymes are faster than those of C3 plants, though they have lower specificity toward CO2 than the land plant enzyme. Replacement of land plant Rubisco by faster bacterial variants with lower CO2 specificity will improve photosynthesis only if a microcompartment capable of concentrating CO2 can also be installed into the chloroplast. We review current information about cyanobacterial microcompartments and carbon-concentrating mechanisms, plant transformation strategies, replacement of Rubisco in a model C3 plant with cyanobacterial Rubisco and progress toward synthesizing a carboxysome in chloroplasts.
Keywords: Nicotiana; Rubisco; Synechococcus elongatus; carbon-concentrating mechanism; carboxysome; chloroplast; chloroplast transformation; photosynthesis; transgenic; transplastomic.
© 2016 The Authors The Plant Journal © 2016 John Wiley & Sons Ltd.
Figures
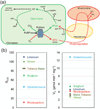
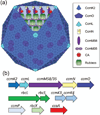
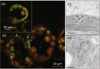
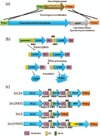
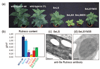
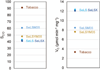
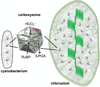
Similar articles
-
Hybrid Cyanobacterial-Tobacco Rubisco Supports Autotrophic Growth and Procarboxysomal Aggregation.Plant Physiol. 2020 Feb;182(2):807-818. doi: 10.1104/pp.19.01193. Epub 2019 Nov 19. Plant Physiol. 2020. PMID: 31744936 Free PMC article.
-
Transgenic tobacco plants with improved cyanobacterial Rubisco expression but no extra assembly factors grow at near wild-type rates if provided with elevated CO2.Plant J. 2016 Jan;85(1):148-60. doi: 10.1111/tpj.13098. Plant J. 2016. PMID: 26662726 Free PMC article.
-
β-Carboxysomal proteins assemble into highly organized structures in Nicotiana chloroplasts.Plant J. 2014 Jul;79(1):1-12. doi: 10.1111/tpj.12536. Epub 2014 Jun 9. Plant J. 2014. PMID: 24810513 Free PMC article.
-
A carboxysome-based CO2 concentrating mechanism for C3 crop chloroplasts: advances and the road ahead.Plant J. 2024 May;118(4):940-952. doi: 10.1111/tpj.16667. Epub 2024 Feb 6. Plant J. 2024. PMID: 38321620 Review.
-
Improving photosynthesis through the enhancement of Rubisco carboxylation capacity.Biochem Soc Trans. 2021 Nov 1;49(5):2007-2019. doi: 10.1042/BST20201056. Biochem Soc Trans. 2021. PMID: 34623388 Review.
Cited by
-
Proteomic changes orchestrate metabolic acclimation of a unicellular diazotrophic cyanobacterium during light-dark cycle and nitrogen fixation states.bioRxiv [Preprint]. 2024 Jul 30:2024.07.30.605809. doi: 10.1101/2024.07.30.605809. bioRxiv. 2024. PMID: 39131303 Free PMC article. Preprint.
-
Engineering photosynthesis, nature's carbon capture machine.PLoS Biol. 2023 Jul 11;21(7):e3002183. doi: 10.1371/journal.pbio.3002183. eCollection 2023 Jul. PLoS Biol. 2023. PMID: 37432955 Free PMC article.
-
The pyrenoid: the eukaryotic CO2-concentrating organelle.Plant Cell. 2023 Sep 1;35(9):3236-3259. doi: 10.1093/plcell/koad157. Plant Cell. 2023. PMID: 37279536 Free PMC article.
-
Engineering α-carboxysomes into plant chloroplasts to support autotrophic photosynthesis.Nat Commun. 2023 Apr 25;14(1):2118. doi: 10.1038/s41467-023-37490-0. Nat Commun. 2023. PMID: 37185249 Free PMC article.
-
Current Metabolic Engineering Strategies for Photosynthetic Bioproduction in Cyanobacteria.Microorganisms. 2023 Feb 11;11(2):455. doi: 10.3390/microorganisms11020455. Microorganisms. 2023. PMID: 36838420 Free PMC article. Review.
References
-
- Badger MR, Hanson D, Price GD. Evolution and diversity of CO2 concentrating mechanisms in cyanobacteria. Funct. Plant Biol. 2002;29:161–173. - PubMed
-
- Badger MR, Price GD. CO2 concentrating mechanisms in cyanobacteria: molecular components, their diversity and evolution. J. Exp. Bot. 2003;54:609–622. - PubMed
Publication types
MeSH terms
Substances
Grants and funding
LinkOut - more resources
Full Text Sources
Other Literature Sources
Miscellaneous